The Weeds of Change
WRITTEN BY AMY BRAVERMAN
PHOTOGRAPHY BY DAN DRY
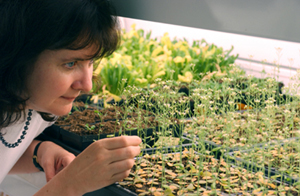
DAN DRY
|
By focusing her research on
a simple mustard plant, geneticist Daphne Preuss is helping to speed
crop improvement—and possibly making some medical breakthroughs.
What if there were an adhesive
stronger than any glue that stuck only to a specific matching element?
What if plants could produce silk as easily as they do cotton? What
if crops could grow in salty soil, resist insects, look and taste
perfect, and perhaps curb allergies too? Or treat cancer?
On the third floor of the Erman Biology Center,
tucked between Botany Pond and the Reynolds Club, the Preuss lab
busily studies the mechanics of such possibilities, all stemming
from a simple weed—the mustard plant Arabidopsis thaliana.
Led by Daphne Preuss, professor in the Department of Molecular Genetics
& Cell Biology, the lab’s 20 or so postdoctoral fellows,
graduate students, and undergraduate assistants examine both the
genetics and the fertility of Arabidopsis, whose small size and
eight-week life cycle make it an ideal biological model—the
plant kingdom’s equivalent of the fruit fly.
Narrowing her research to a foot-long weed with
a straight, skinny stem, stubby leaves, and tiny, white, tubular
petals has paid off for Preuss, at age 40 one of the world’s
top geneticists. These days if she’s not in Italy sharing
her research with fruit-fly experts, she may be in New York addressing
the United Nations about genetically modified crops or in Madison,
Wisconsin, for the International Arabidopsis Meeting. The
wavy-haired scientist with a soft, unassuming voice is in her lab
about two weeks a month—more often in summer—and manages
phone calls as much as lab workers, scans her computer screen more
often than a microscope slide.
Before the mustard plant shot her to the genetics
forefront, Preuss studied yeast as an MIT doctoral student from
1985 to 1990, planning to stick with the fungus as a Stanford postdoctoral
fellow in 1991. But soon after her arrival in Palo Alto she switched
to plants. “There hadn’t been a lot of molecular biology
done on plant cells,” she says. “I also liked that the
distance from initial discovery to real-world applications seemed
shorter.” From the beginning she focused on pollen, which
“had amazing cells with long tubes, was critical to the life
of the plant, and seemed like a complex enough system.”
In 1994, after spending a week examining thousands
of mutant Arabidopsis pollen grains under a microscope,
looking for “ugly pollen, weird things” that might lead
somewhere, Preuss found the mutant that would determine all of the
genetic work she’s done since. Instead of each single pollen
cell sitting on the slide by itself, unattached to others, she saw
four cells sticking together—a tetrad. Such foursomes happen
to be common in Preuss’s former research subject, yeast, and
had been key in helping scientists to trace that organism’s
genes precisely. But because no tetrad mutant had been found in
any plant, the leafy kingdom remained genetically mysterious. (Preuss
later learned that 50 years earlier a scientist had reported a petunia
tetrad, but it was never studied; no others have been reported since
her Arabidopsis find, and as far as she knows no one is
looking.)
The tetrad is important because it allows geneticists
to follow genes after cell division, or meiosis. During normal meiosis
Arabidopsis’s ten chromosomes line up into five pairs,
and the partner chromosomes exchange bits of DNA—a process
called recombination. The chromosomes split from each other as the
cell divides into two, each new cell containing five chromosomes,
one from each pair. Then those chromosomes split in half during
the second cell division, forming four distinct daughter cells containing
five chromosomes each. The daughter cells go their separate ways,
and it’s difficult to track them. But in a tetrad, created
when the daughter cells don’t divide correctly and their cell
walls fuse together, scientists can monitor the cell family closely.
They can follow what happens to each chromosome after recombination,
opening all sorts of genetic doors. “If you can capture how
chromosomes move, you can map part of the chromosome,” Preuss
says, referring to the elusive centromere, considered a chromosome’s
center, where chromosome pairs attach as they swap DNA and where
they split off again. “The day I saw [the tetrad] I knew we
could do just that.”
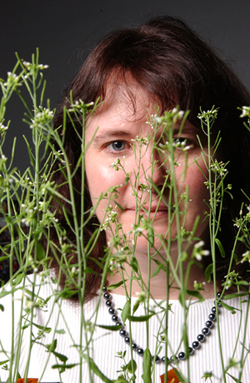
DAN DRY
|
Preuss eyes full-grown Arabidopsis
plants. |
She soon got her chance. In 1995 Preuss joined
the Chicago faculty and began honing in on Arabidopsis—half
of the Preuss lab researched its genetics, while the other half
studied its fertility, a double focus that continues today. The
National Science Foundation began a six-lab Arabidopsis
genome project, with the Preuss lab responsible for mapping the
plant’s centromere. Many supposedly complete genome projects
leave out the centromere’s gene sequence because scientists,
without the benefit of tetrad studies, rarely can locate it. While
genes along a chromosome’s arms get exchanged during recombination,
the centromere stays intact. So in tetrads, where researchers can
follow recombination, they can narrow down the centromere, locating
where along the chromosome less and less recombination takes place.
Preuss lab postdoc Greg Copenhaver examined 1,000 meioses before
he and Preuss decided they had enough data to pinpoint the location
and gene sequence of Arabidopsis’s centromere. Their
findings were published in the December 1999 Science.
Once Preuss knew the coding for all five Arabidopsis
chromosomes, she could recreate them. With postdoc Kevin Keith she
began producing “minichromosomes”—composed of
the centromere, telomeres (chromosome ends), and only about four
genes rather than the bulky 5,000 on a natural Arabidopsis
chromosome. Preuss can put any type of gene she wants on a minichromosome,
and she imagines increasing the number to about a dozen. So far
she’s made protein cells glow fluorescent green under UV light,
“to use as a marker to see which cells contain that chromosome,”
and she’s made Arabidopsis resistant to herbicide.
“These are just test cases,” Preuss says, but real-world
cases may arrive soon.
Shortly before the entire genome project’s
results were reported in the December 2000 Nature, Preuss,
Copenhaver, and Keith founded Chromatin, Inc., a Chicago-based start-up
that has licensed the technology to identify a centromere and build
a chromosome. With this technology Chromatin, named after the DNA
proteins that make up chromosomes, plans to speed the pace of crop
improvement. Currently geneticists can insert one, maybe two new
genes at a time into a plant chromosome to change an attribute:
they’ve created crops containing their own pesticide or herbicide,
for example. But it’s a limited, time-consuming process. To
insert one gene they must grow 1,000 plants, hoping that in one
or two the gene lands on the correct chromosome spot to create the
desired result. For a second gene they must go through the process
again. Often the genome gets damaged in the process, and the scientists
must “perform selective breeding for a few generations to
clean the genome,” Preuss says. “All these current genome
projects are identifying lots and lots of genes, but there are only
two or three new products a year” in the agricultural industry.
On Preuss’s artificial minichromosomes
“you’ve built the genes’ environment around them,
and they’re all inserted in a single event,” meaning
a geneticist can tweak many plant characteristics at once. And though
a 2001 New York Times article conjured up “a cucumber
that resists both cold and drought, produces its own fungicide,
herbicide and pesticide, has electric blue skin (or orange or mauve),
tastes like barbecue sauce and grows to the exact circumference
of a McDonald’s hamburger, so that it makes a perfect-sized
pickle,” Preuss has both playful and noble ideas. (Named a
Howard Hughes Medical Institute Assistant Investigator in 2000,
she’s prohibited from endorsing companies or products, but
she can speak generally about the kinds of developments that could
be made by companies such as Chromatin, which she advises and in
which the University holds shares.) One “amusing thought experiment”
is the possibility of plants producing silk. “Given enough
time the technology could get there,” Preuss says. “Silk
would probably become as cheap as cotton, and I know my own wardrobe
would be different.” On the weightier end, she considers inserting
medicines into plants. The cancer treatment Taxol, for instance,
comes from the Pacific yew tree. Chemists can make the drug, but
it’s a difficult process. “It turns out the yew tree
uses 14 genes to make Taxol. If you could take those 14 genes and
put them in a soybean, that would solve that problem.” As
a bonus, growing medicine or vitamins in a crop minimizes lab or
factory pollution.
Because of its ability to speed crop improvement,
Preuss believes, the minichromosome technology could also help relieve
world hunger. One already solvable issue concerns salt, which contaminates
30 percent of the world’s irrigated land, rendering it unfarmable.
Inserting a specific gene permits crops to grow in salty soil, she
says, and could help feed starving people around the world. But
genetically enhanced crops face stiff opposition, especially in
Europe, which is why Preuss addressed the United Nations last November.
“The leading cause of death worldwide is starvation,”
she says, citing a 2002 World Health Organization report. “We
have the technology to make crops better, but it’s not being
done because of fear. Most of the land in the world is not ideal
for agriculture, and as the population increases there aren’t
a lot of options. We can either cut down the rain forests”
or alter crops for less friendly soil.
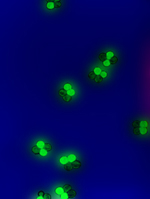
Tetrad by
DAPHNE PREUSS
|
The tetrad mutant (left) that opened
genetic doors. |
Humankind has been tinkering with genes for millennia,
she points out, through crossbreeding. Modern maize, for example,
didn’t start out as the lush corn kernels we eat today. The
original plant produced few seeds, each encased in a tough shell.
Central and South American people 10,000 years ago discovered mutants
that made the plant better, exchanged those mutants with other communities,
and eventually got maize. With the emergence of modern genetics
about 100 years ago, crossbreeding accelerated as biologists purposely
created mutants by exposing plants to radiation or chemicals, creating
more diverse, more resistant crops. Although that practice was seen
as merely an extension of conventional breeding, when molecular
biologists stepped in, fear grew.
After her U.N. speech, Preuss says, an African
ambassador whom she prefers not to name told her that she’d
changed his mind about the issue. The Zambian ambassador, meanwhile,
told her that activists had warned that genetically modified food
would make people impotent. “This was corn that had been on
the U.S. market for years,” Preuss says. “It’s
all scare tactics.” She doesn’t mind if food-rich European
countries don’t want genetically modified crops. “But
they shouldn’t deny the rest of the world the chance”
to grow needed plants. Soon the world court will decide the issue:
in May the United States filed a dispute with the World Trade Organization,
claiming the European Union's five-year moratorium on accepting
genetically modified crops unfairly blocks exports from American
farmers.
Preuss’s Arabidopsis advances
don’t stop at plants. Meiosis occurs the same way in plants
as in animals, so following mustard-plant tetrads also can provide
insights into human biology. She’s currently studying how
chromosomes get lost during meiosis. As women age, for instance,
they lose chromosomes from their eggs. In older women who become
pregnant, therefore, the chromosomes are more likely to divide incorrectly,
sending two copies of a chromosome to one cell and none to another.
If two copies of Chromosome 21 get captured in an egg, and then
sperm fertilizes it and adds a third, the child will have Down’s
syndrome. Testing different drugs on the pollen cells to see how
they affect chromosome loss, she hopes that one day a medication
may help solve such problems.
Pollen cells, examined
so thoroughly in Preuss’s genetic studies, carry the
plant’s sperm, making them equally valuable to the lab’s
other half—the fertility researchers. In Arabidopsis’s
white flower’s center, powdery, yellow pollen cells are formed
by the plant’s two long, male anthers. At the anther’s
top the pollen falls onto the female organ’s tip, or stigma.
There a pollen cell sprouts a tube, which travels down through the
ovary until it finds an egg cell to fertilize. The Preuss lab studies
plant mutants that distort every stage of the pollen’s journey,
trying to understand what attracts pollen tubes to eggs.
Using confocal, electronic, and atomic-force
microscopes, postdoc Anna Edlund photographs the process—the
pollen tube emerging, its location one minute later, two minutes
later, and so on. She wants to know how the tube breaks out of the
cell wall, a structure so resistant that pollen grains survive for
millions of years inside fossils. “In some mutants I’ll
see tubes that are confused and grow the wrong way,” Edlund
says. It’s yet another irregularity shedding light on a regular
process—this time what signal tells a pollen tube which direction
to travel.
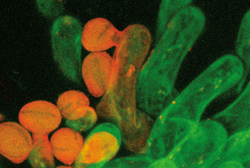
Stigma by ANNA EDLUND
|
Green dyed Arabidopsis stigmas dusted
with red pollen grains |
Ravishankar “Ravi” Palanivelu, a
four-year Preuss lab postdoc, blends the lab’s genetic and
fertilization studies, trying to figure out which genes and chemicals
are responsible for pollen-tube growth. Growing Arabidopsis
on petri dishes and applying different chemicals, Palanivelu discovered
that the GABA neurotransmitter—also central for human nerve-cell
communication and linked to epilepsy and mood disorders—helps
lead pollen tubes to eggs. “I never imagined that such a molecule,
already known in humans, would be so important in plants,”
says Palanivelu, whose research appeared in the July 11 Cell.
Preuss also studies how a pollen cell attaches
to a plant’s stigma—moreover, why pollen, after blowing
in the wind or latching onto bees, binds only to matching female
species. This project dates to 1997, when the Preuss lab put different
types of pollen on Arabidopsis flowers and then washed
them with hard salts, detergents, and other substances. The foreign
pollen fell off, while the related cells stuck. Not only did they
stick, she discovered, but they fastened to the female like the
toughest Velcro. Last year the lab found mutants in which related
pollen didn’t stick, which will help the researchers learn
which genes affect the binding—and perhaps bring them a step
closer to creating an adhesive that makes superglue look like Scotch
tape. “Most glue sticks to anything,” Preuss says. “With
this [plant adhesive] you could coat a surface with the male and
then nothing would stick unless it was coated with the female.”
Such a glue could make building tiny devices, such as nanomachines
and micromachines, less clumsy. Or, jokes physics professor David
Grier, who helped Preuss measure the pollen bond’s strength,
if an envelope were coated with the female and a stamp with the
male, stamp licking would become obsolete. And all plants, not only
Arabidopsis, work this way. Researchers could play with
different varieties to see what works best.
What’s worked best for Preuss has been
the small Arabidopsis weed—allowing her to sculpt
chromosomes, study disease, perhaps feed the hungry. “There’s
a huge universe of possibilities you could do,” Preuss says.
“What do you do first?” As Preuss keeps experimenting
and Chromatin gets off the ground, the world may soon find out.
|
|